If we possess our why of life we can put up with almost any how.
Friedrich Nietzsche
Overview
We both build and compose all the parts of our swarm, but we don’t intend everything that happens when those parts work together. It can do things that we don’t expect, don’t like, or don’t even understand. But it doesn’t merely act randomly. It sometimes seems to act as if it’s willful—at least in the sense of being driven by internal dynamics that appear patterned, but that are different from anything we planned. Could it be somewhat like a living thing? Trying to answer that question brings up the definition of life, which involves reexamining all ten swarm-physics ideas introduced thus far: ‘reaction networks’ (chapter 2), ‘autocatalysis’ (chapter 1), ‘closure’ (chapter 5), ‘synergy’ (chapter 2), ‘autopoiesis’ (chapter 5), ‘recursion’ (chapter 3), ‘phase change’ (chapter 1), ‘stigmergy’ (chapter 3), ‘ecogenesis’ (chapter 4), and ‘non-linearity’ (chapter 4). If a network could grow so complex that it acts so as to preserve itself against dissolution, it might make sense to say that it is, in some sense, alive.
Sparks of Life
Is our swarm alive? Many biology texts follow Aristotle, our first major biologist, in saying that living things are those things that breathe, eat, grow, reproduce, and such, so in that sense our swarm surely isn’t alive. But such a definition has problems. A mule can’t reproduce, yet we say it’s alive. Fire can reproduce, but we don’t think of it as alive. An emperor moth can neither eat nor grow, yet we accept it as alive.
Other biology texts avoid such problems by saying that living things are gooey masses made of cells, each of which has the same genes, while some texts say that living things maintain their own internal state, and evolve. Again, by either of those definitions, our swarm isn’t alive. But such definitions also have problems. A virus has genes and can evolve, but has no cells. Is it alive? A prion has no genes or cells, but can copy itself. Is it alive? A slime mold spends most of its time as separate foraging cells. Is it alive? A Portuguese Man o’ War looks like a jellyfish but it’s really a colony of cells from four different species that join together into one mass. Is it alive?
Aristotle’s ideas about such questions are still worth thinking about today, for versions of his thought are still current among many of us. So imagine that we could resurrect him, hand him a microscope, and ask him to watch a microbe as it moves around on a petri dish that has some sugar sprinkled on it. If the microbe starts off in a sugar-poor area, it will bop around until it ends up in a sugar-rich area. If we then ask him how it did what it did, what might he say? Since he’s never seen a microbe—or a microscope to see it with—he would have to consider the question afresh.
After he brushed the soil from his rotted toga—and combed over his bald spot—he might say something like this: “It doesn’t act at random, like smoke buffeted by every passing puff of air. It also doesn’t act in a fixed way, like a boulder falling off a cliff. I also don’t see anything prodding it along to make it act the way it does. It thus looks as if it’s self-willed, like a hummingbird darting from flower to flower, or as you or I might, were we seeking a lost donkey in a dense olive grove. So it must have an unseen, built-in drive to seek sugar. In short, it loves sugar.” That is, ‘loving sugar’ is one of its built-in tendencies.
Aristotle, like many of us, thinks in terms of ends—goals, aims, intents, purposes. So, for him, something inside the microbe must be driving it to seek sugar. Also, for him, that something must have been put there for that purpose. But since he has no idea how the insides of the microbe work, he may be assuming that it’s like him and thus that it’s behaving in a goal-driven or purposive way.
Further, his ‘it-loves-sugar’ answer isn’t an answer to the ‘how’ question we asked him. It’s an answer to a ‘why’ question he made up, namely: “Why did the microbe move from a sugar-poor area to a sugar rich area?” Also, ‘loving sugar’ is less an answer to his made-up ‘why’ question than it’s a confession of his ignorance about the ‘how.’
So we hire some molecular biologists who try to work out the how. They descend to the cellular level and tease apart the microbe to see how it moves, eats, excretes, and such. After decades of work, they discover that it has 4,357 genes, and together with some small signaling molecules, those specify and control the proteins that react together to let it do what it does. To a first approximation, the microbe seems to be nothing but a bag full of many copies of proteins, plus a description of those proteins. It’s a bag holding a reaction network of genes and proteins. That’s all. It needs no ‘desire’ for sugar.
‘Microbes love sugar’ is a simple, but misleading, answer. It suggests a tiny thing, with an even tinier brain, scheming how to get sugar. But even if that were true, it wouldn’t say much about microbes because all it’s really saying is that the real cause of the microbe’s actions must be in its alleged brain, and we wouldn’t know how that works, or even that it exists. So the statement amounts to saying this: “If a lot of us who don’t know anything about microbes look at one through a microscope, here’s what most of us might say when we try to explain why it’s doing what it’s doing.” That’s more of a statement about how we think than about how microbes work.
However, after we work out the how of what a microbe does, we learn many things. That might help us make new foods and fuels, prevent disease, make things that continuously make and dispense medicines inside our bodies, and better understand not just microbes but also our bodies. That new insight might change the world. But it doesn’t give us any suggestive image. It doesn’t say why microbes do what they do. It merely says how.
That might disappoint our reincarnated Aristotle because he’s driven to seek ‘why,’ not ‘how.’ He believes that if he can guess the why of something then he can deduce its how, whereas knowing its how needn’t tell him anything about its why.
So he’s now likely to ask another ‘why:’ If the microbe wasn’t purpose-built to do what it does, why then does it exist at all?
Explaining to him how today’s microbes may have come from yesterday’s microbes might boggle him, but won’t fully answer his question. Evolution explains a lot about how living things change, but not the origin of life itself. Sketching how the microbe’s chain of ancestors may have passed the spark of life from link to link backward across billions of years just leads to some first cell. So after we explain everything we know about that chain, he might then point out that no matter what else that first cell may or may not have had, it most likely must have had a metabolism. That is, it must have had some way to extract matter and energy from its surroundings. If it couldn’t do that, it couldn’t live. Why, then, did that come to be?
Again he’s asked a why, and we can’t easily answer. So we hire some biochemists who try to work out the how. They descend to the molecular level, inside the microbe, and spill chemicals in labs. After decades of work, they say that the key seems to be catalysis. A catalyst raises a chemical reaction’s speed, often by millions of times, while itself remaining unchanged. It’s a helper.
All known cells need catalysts. For instance, nearly every cell in our body needs thousands of copies of thousands of proteins and without them we couldn’t extract oxygen from air, nor digest food, nor grow, nor do anything else. Those proteins build things, break things, move things, sense things, signal things, and on and on, and many of them are catalysts. Those catalysts are highly specific to the reactions that they aid. So a cell, by making a particular catalyst at a precise time, can turn on a specific reaction just when it needs that reaction.
That precision lets cells take energy from one place, move it to another place where it usually doesn’t want to go, store it there, then release it where and when it helps them persist. That control over energy buildup, storage, and release lets cells stitch atoms to, or cut them from, other atoms. That atomic engineering lets them shape matter, and maintain such shaped matter, to thus continue to shape matter and control energy, and thus keep going.
Cells do all that engineering by making sure that they always have enough copies of certain specific molecules. Take adenosine triphosphate (ATP). A small molecule, made of just 47 atoms, cells use it like a rechargeable battery to store and transport the energy packets they need when stitching together or cutting apart molecules (and doing just about everything else). But cells don’t make lots of copies of it all at once then try to store them; those copies would quickly vanish. (The average cell uses up all its ATP in a few seconds.) Molecules, especially the unstable or high-demand ones that cells need, needn’t last long. So for a cell to persist, it’s cheaper and safer to have lots of fast reactions that can quickly make the stuff it needs—and only when needed—out of parts that it has or can fetch from its surroundings.
One such type of fast reaction is autocatalytic (‘self-helping’). Such reactions catalyze themselves by making something that itself speeds them up. For example, imagine two molecules named Watson and Crick. Suppose a Watson can use ATP to help it take some parts and make a Crick, and similarly a Crick can use ATP to help it make a Watson out of some other parts. If we put a Watson and a Crick in a bucket of water full of the right parts plus lots of ATP, the Watson could crank out more Cricks, and the Crick could crank out more Watsons. But since a Crick makes a Watson, which makes another Crick, Crick’s reaction catalyzes itself—it’s autocatalytic.
If a cell needs Cricks (or Watsons) that’s a useful kind of reaction for it to have, but what if it can’t make something else it needs? Sure, it could make ATP from sugars, but suppose it doesn’t have, or can’t get, any sugars? Well, it might still be able to make ATP by first breaking down larger carbohydrates or even fats. To do so, it would need more reactions, and not all of them may be autocatalytic. How might it ensure that it has all those reactions ready to go if conditions change? And how can it ensure that those reactions will be fast enough?
Autocatalytic reactions help themselves persist directly, but other reactions can help themselves persist indirectly. For instance, imagine two more molecules, Holmes and Moriarty. Suppose a Watson can make a Holmes, which can make a Moriarty, which can make a Crick.
Watson → Holmes
Holmes → Moriarty
Moriarty → Crick
How might a Crick help ensure that copies of itself persist? That is: What makes more Cricks? Well, any Watson (or Moriarty) would. So if a Crick helps a Watson (or Moriarty), it would help itself. But so would helping a Holmes, since it makes Moriarties, which make Cricks. Even Cricks themselves do, since they make Watsons, which make Cricks (or Holmeses, which make Moriarties, which make Cricks).
Thus, if a Crick does anything that helps a Watson, a Holmes, a Moriarty—or even another Crick—it would help Cricks persist. That’s true for all four molecules. The network that they belong to lets each one help all the others persist. They don’t need any built-in desire to help each other persist; they still will, simply because of their network’s structure.
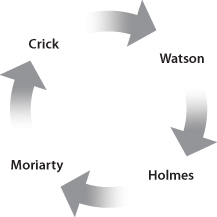
Crick helps itself persist by helping Holmes persist
If, in any reaction network, every reaction’s catalysts are made by other reactions in the network, then the network as a whole has catalytic closure. It remakes all its own catalysts. Given enough parts to work on and enough energy to work with, it’s a self-persistent set of reactions. Such reaction networks are synergetic (‘jointly self-helping’).
Autocatalysis is the special case of synergy where there’s only one (self-)helper reaction in a network. Further, autocatalysis needn’t be limited to the molecular world. During our farming phase change, some of us plus wheat equaled more of us plus more wheat plus tools. Synergy, too, needn’t be limited only to molecules. During the second wave of our industrial phase change, coal mines plus iron mines plus steam engines plus factories plus railroads meant more mines, engines, factories, and railroads. That, too, was a synergetic network, as was an earlier one involving paper mills and printing presses.
In sum, looking at life-forms through the lens of reaction networks rather than breathing and such, or genes and things, suggests a different way to define life. Instead of focusing on what living things do (their behavior) or what they’re made of (their substance), perhaps it’s useful to consider redefining them in terms of how they work to help themselves persist (their structure).
Diversity and Density
Our reborn Aristotle might well accept various ideas about reaction networks and catalysis and such. But he might now say: “Aha! But why do just the right set of co-catalyzing molecules fall together into a self-helping reaction network in the first place? That can’t happen at random. That would be like stuffing the pieces of a jigsaw puzzle into a box, shaking the box, and having them assemble back into the jigsaw’s picture. Thus they must somehow have some built-in urge to help each other.” In short, he might now try to move his assumption of built-in purpose down from a cell to the molecules that compose it.
The regenerated philosopher has a point. Were synergetic reaction networks to fall together at random out of random parts, why are they so highly non-random that they are self-helping? Wouldn’t they need some sort of unseen driver or guiding hand or some such thing to select them from all possible parts so that their parts will then react together in the intricate ways that they do? Again, evolution doesn’t answer his question because it doesn’t apply to the origin of whatever the first life-form may have been. And again our last ‘why’ has turned into a ‘how,’ then turned into another ‘why.’
So now we hire some mathematicians and computer scientists who try to work out the how. They ignore test tubes and microscopes—and cells and genes—and even molecules and atoms. Instead, they build statistical models and computer simulations and head off into abstraction. They drink coffee, scribble theorems, and bicker. Then—after the usual decades of work—they show that if they start with any complex enough reaction network, then some subset of it will, almost surely, become self-helping. But they can’t guarantee that. Nor can they tell ahead of time which subset that will be.
However, what they can say is this: all that the original network needs is a constant flow of matter and energy, plus two simple properties: It must be both very diverse and very dense.
Here’s why: Every molecule is in constant danger, whether from the wear and tear of random collisions or because something is trying to eat it, destroy it, use it, or build on it. Its copies, if left to themselves, will always die out. So if a cell has to rely on any molecule, that cell has to be ready to make lots of copies of that molecule.
Think of a molecule like a part made in a factory, and a chemical reaction like a factory that takes in specific parts and pumps out other specific parts. If copies of any molecule are to persist in a reaction network, that molecule needs a factory (or factories) making more copies of it. But such factories, if they themselves aren’t to die out, will need parts as input. Those inputs must come from yet other factories as output, and so on.
Imagine we have a factory that’s supposed to pump out hacksaws. To make hacksaws, it needs saws and frames and nuts and screws as inputs. So some factories need to make those as outputs. Those factories might need hammers and vises and such, as inputs, which other factories must make as outputs. Then suppose that some other factories, given hacksaws, and drills, plus other inputs, could make electric motors, which yet other factories could use as inputs, together with other inputs, to make drills, and many other things, perhaps even including hacksaws. The whole production system could thus close in on itself.
But, hang on, why must that work? Couldn’t it mean that the persistence of copies of just one part might need an infinite set of factories? Well, yes, but in complex enough reaction networks, closure could come to the rescue. One factory dependent on other factories, which are dependent on yet other factories is like looking up a strange word like ‘fnord’ in a dictionary and seeing other strange words, like ‘hoopy’ and ‘discordia,’ in its definition. Then, on looking up those, finding ‘frood,’ ‘bogon,’ and ‘kluge’ in their definitions. That could go on forever unless, after a while, we run out of strange words. (So that—even if we keep seeing lots of them—at least no new ones show up, so they’re all defined in terms of each other.)
That is, the inputs that many factories in a cell need may overlap a lot with the outputs that many other factories in the cell make. It then becomes a numbers game. If the number of factories is large enough, then, statistically speaking, it becomes more and more likely that there could be some subset of those factories that could make all the parts that the same subset needs. That subset could then help itself. That is, its factories could together create all the parts that its factories together need.
Such a self-helping subset isn’t guaranteed to exist; but if it does, then all factories that aren’t in it must die out. At least one of the parts that any such factory (outside the self-helping subset) needs as input won’t get remade by some factory as output.
Further, although the size of that self-helping subset might be far smaller than the original set, it might still be quite large—because each of its factories might need many parts, each of which may need many factories to make them. So if a reaction network is to persist, it might need many kinds of factories—that is, it may need to be very diverse.
Also, since no part lasts forever, distance can matter. (‘Distance’ here is defined in terms of how fast parts can move between factories relative to how fast they decay or are used up.) For any factory in the self-helping subset, a part is useful only if it gets from where it’s made to where it’s needed before the amount of it falls below some threshold. Thus, the distances between all those factories must be short. Thus the subset of self-helping factories must be very dense.
So if we start with a widely diverse set of factories (that is, if they can make ‘a lot of’ different parts), and they are very dense (that is, if they are ‘close enough’ to each other), and they are surrounded by ‘enough’ energy and spare parts, then they seem likely to form a synergetic subset of factories, and thus might be likely to persist.
The power of diversity coupled with density needn’t be limited to the molecular world. Innovators in Britain in the 1700s had many different and complementary early industrial skills. (They were diverse.) But besides that, they could work as a unit strong enough to trigger the first wave of our industrial phase change partly because they were also squeezed together. (They were dense.) They together formed a subset of the whole, but that subset was self-helping. (They didn’t need to be self-sacrificing, just self-beneficial—that is, synergetic.) Many of them knew each other. Many of them had similar beliefs and inclinations. Plus, they all could read and write. So they could learn about each other and talk to each other. Also, thanks to repression, several of them had no choice but to work with each other. So, often, what one needed, others made. In a way, they were like ‘factories.’ And the ‘distances’—in terms of transport of both matter and data—between them were ‘small enough’ that anyone could put to use anyone else’s output before it ‘decayed.’ (In part, that’s why earlier inventions like the printing press were so important—they shrank ‘distance.’)
In short, those innovators could ‘trade’ their new ideas and devices fast enough for it to matter. Thus the work of each of them could build on each other’s work before dying out. One and one could thus make not two but three: 1+1=3. Take away either their diversity or their density and the synergetic network that they stitched themselves into may never have happened.
Similar things might be said about a big city versus a small village—and now that we have rapid planetary transport of matter and data—about a whole planet versus a small country.
Of course, all of the above is just math and guesswork. It’s just what models suggest might be true, not what labwork demonstrates must be true for real molecules in real test tubes. But it does suggest that synergy is likely in some subset of any sufficiently diverse and dense network. And if diversity couples with density in a sufficiently energy- and parts-rich surroundings, something vaguely like the beginnings of life might result. Maybe.
Entelechy Shmentelechy
Our revenant Aristotle might well accept that density coupled with diversity may possibly lead to synergy. After all, he thinks that many living things spontaneously self-generate in a ‘frothy bubble’ of earth, water, air, and ‘vital heat.’ For him, that explained why flies sprang from rotting meat, eels from mud, oysters from slime, cockles from sand, hermit crabs from soil, mistletoe from tree branches, bees from flowers.... Lacking optical aid, he couldn’t otherwise explain how such things reproduced. Plus, he lived in a world made of just four elements—earth, water, air, and fire—so they had to explain everything. So pressing him for details on how such ‘frothy bubbles’ form would probably just lead to him talking in capital letters: Entelechy, the Life Essence, the Vital Principle, the Breath of Life, the Soul. As usual, he’s seeking the ‘why,’ not the ‘how.’
He isn’t being evasive. He’s trying to separate the matter making up a life-form from the arrangement of that matter making it the kind of thing it is. (We today make a similar sort of distinction when we try to separate matter and information—and more recently, network structure and network dynamics.)
Thus, if we hand him a crab he might say that it’s a mass of crab-stuff: claws and a shell and such like. But, as he’s quick to point out, that’s just its matter. That’s far from all that matters. For were he to take a hammer and break its crab-stuff into bits, no matter how small, that wouldn’t tell him what a crab is; it could only say what it’s made of. For him to call something a crab, that crab-stuff must also have crab-actions: it must use its claws and shell and whatnot to scuttle and eat and grow, and so forth. For him, something must give the crab those capacities. That something is the essence of crabness; the soul of a crab.
His use of the word ‘soul’ is subtle. He doesn’t mean something that lives on forever. He’s trying to distinguish between a puppet and its performance. Whatever is causing the puppet’s animation can’t be like a puppeteer—for if it were, it itself would then be made of matter, and what would animate it? So by itself it can’t be the crab. Whatever it is, it needs crab-stuff to ensoul to thus give that crab-stuff the capacity to do crab-things. But the crab-stuff alone can’t itself be the crab, either; it needs a crab-soul to animate it.
So, for him, each crab must be made of two things: crab-stuff and crab-soul—one physical and one non-physical—and they aren’t separable. When one dies, the other dies with it. In short, for him, every living thing has a soul, and everything with a soul is alive.
Thus, even if he accepts ideas about diversity and density, he’s likely to cling to his idea of entelechy, which means something like: ‘having one’s purpose inside oneself,’ or ‘being at work to keep oneself in being,’ or ‘working to remain oneself.’ He might thus still argue that living things must have built-in purposes, because life is more than mere density and diversity. For instance, a cell isn’t merely self-helping; it’s also self-contained, and the two are connected. So he would say that it works to maintain its own containment because without containment it can’t maintain its density and diversity. It works to remain itself.
Again the revivified philosopher has a point. How could something come to maintain its own containment?
Imagine trying to build a desktop model of a cell, where each reaction is like a (small) factory, and each molecule is like a factory part. But these factory parts aren’t simple doodads, like nails, screws, nuts, or bolts. Think of one as being made of hollow plastic that’s lightly coated in steel, about the size of a fingertip, and of some convoluted shape. Inside it is a tiny battery, and scattered over its surface are several tiny push-button switches and tiny electromagnets. Each switch controls some electromagnet.
If we stuff a bunch of such parts in a big duffel bag and shake the bag for a while, the parts will bump into each other. Two such parts might bump into each other in such a way that they press some of each other’s switches and so magnetically lock together. Their shapes, and where their switches and magnets happen to be, will determine how many of them might lock together per second. (Chemical reactions are faster and more intricate, but that’s the general idea.)
Now to make a model of a cell, start with at least several thousand different such parts. (Just a few hundred may not do. That may not lead to a diverse enough set of reactions.) Stuff them into a bag that has small holes at its top and bottom. Stick the bag’s top under a faucet and let water jet in. The rush of water will then tumble the parts around. Some of them might then lock together. Then some of those joined parts might lock together, and so on. If we start with a big enough bag, strong enough water pressure, and enough different parts, a few such combined parts might grow quite big.
Among such big parts, two kinds that might occur are very special.
One kind is a cutter. It may happen to be so shaped that it can latch on to a particular big part, and by doing so, its shape changes. That shape change presses buttons on both it and the engulfed part in such a way that it breaks locks between two of that part’s pieces, so they separate. That separation presses buttons on the cutter that return it to its old shape, which ejects the pieces, like a toaster ejecting bread. So from big parts it makes smaller parts, while remaining the same.
Another kind is a stitcher. It may happen to be so shaped that it can engulf two particular small parts, and as it does so its shape changes. That shape change presses buttons on both it and those two parts so that they snap together, forming a larger part. That formation presses buttons on the stitcher that return it to its old shape, ejecting the new part. So from small parts it makes bigger parts, while remaining the same.
If they arise, cutters and stitchers are like little robots that patrol the bag. They do their job if they happen to bump into the right parts in the right way. Just based on their shape—with no eyes and no hands, and no brains—they break down and build up other parts in the bag while themselves remaining unchanged. They’re catalysts.
For example, in many cells, sucrase (a catalyst) breaks up sucrose (a sugar) into two other sugars—fructose and glucose. It’s a cutter. However, sucrose synthase (another catalyst) joins those same sugars back into sucrose. It’s a stitcher. To persist, the cells we today know of need at least a thousand different kinds of cutters and stitchers.
But even if such a sophisticated thing were to arise by chance, what would possibly let it persist? If we were to follow any particular part as it bops around in the bag, it must, at some time, cease to exist in the bag. Either it’s jostled too much and falls apart, or it’s cut apart, or it’s stitched into other parts, or it falls out of the bag with the escaping water. However, copies of it might still continue to exist in the bag. How? Even though every single part will surely vanish at some time, copies of it might remain in the bag for quite a while—if other parts make enough of them fast enough.
Imagine a troupe of circus trapeze artists performing without a net. The law of gravity and the chance of mistake will ensure that all of them must, at some time or other, fall to their death. But the troupe as a whole may persist for a long time if, for any particular one, others make copies of it in midair as they fly from trapeze to trapeze—if yet others are making copies of those in midair as they fly from trapeze to trapeze, and so on. In that way, copies of one such artist can remain tumbling in the bag if copies of all other such artists are presently tumbling in the bag, and if they react together often enough to keep making enough new copies of it.
Thus, if there are a set of parts in the bag that can react together not just synergetically but also fast enough compared to the water’s speed, they have a chance to persist in the bag. And if we start with a diverse enough set of parts, and if the bag keeps them dense enough, and if the water keeps them tumbling enough, some subset of them might have chance to become synergetic. Over time, all the rest will flush out.
However, the reaction network that the bag contains may have more than that catalytic closure (that is, it may be more than merely synergetic; that is, jointly self-helping). It may be resource-capturing, too.
If a contained network is so structured that once it has enough parts and energy trickling in, it acts to fetch more—it has operational closure. Such a network is more likely to persist than one with catalytic closure alone because the interlinking of its parts and reactions locks in most of its resource supplies. As useful parts filter through the bag they’re captured and used up in the network’s reactions, and useless parts are flushed out of the bag.
In sum, for the network to have any chance of persisting at all, it needs energy and parts. It needs parts to capture energy from the energy flow. And it needs more parts to replace and repair parts lost doing that. To make the first work, it needs to have catalytic closure; and to make the second work, it needs to have operational closure. And it needs to be inside a bag to keep all its parts together.
At this point, Aristotle interrupts with a question: Where might such an increasingly unlikely network’s enclosing bag come from? Well, the bag may itself be made of parts. The reactions that the bag encloses make parts, so they might even make bag parts. If some reactions do, and if other reactions stitch those parts together to maintain the bag, then the whole network might then maintain itself. Why? Well, its reactions would be contained in a bag because they would make the bag. The bag would keep together because the reactions inside it would keep working. Those reactions would keep working because they’re being kept together by the bag, which they themselves would maintain. Everything would then depend on everything else, the bag included. Otherwise it’s like a cup of water hanging in the air, minus its cup.
Of course, for the network to keep making and stitching together bag parts it’s going to need a continuous inflow of parts to make those bag parts. And to do all that stitching it’s going to need a continuous flow of energy, which it gets from a continuous inflow of water that keeps those parts bumping into each other.
It thus needs a constant inflow of matter and energy. (It can’t exist, and won’t persist, unless it’s in an energy gradient, where energy flows from high concentration to low concentration, just as a waterwheel is pointless unless it’s in a river, or a windmill won’t work on a calm day. All living things exist by sipping from some energy flux, like slinkies falling down an infinite staircase.)
If a network has catalytic closure (that is, it’s synergetic), and it has operational closure (that is, it’s self-remaking), and it’s contained in a bag that it itself maintains (that is, it’s self-containing), it is autopoietic (‘self-maintaining’). Once given a continual flow of enough of the right parts, and a continual flow of the right amount of energy, it has a chance to preserve its density and diversity. That preserves both itself and its bag.
A synergetic network can persist itself—if something keeps it contained. An autopoietic network, though, because it can keep itself contained by remaking its own bag, can keep itself going. It’s fully recursive.
All known cells must maintain a barrier between themselves and the outside. Only so can they concentrate the molecules that they need to ignite the chemical reactions that they need and remove those that they don’t. They do that with a cell wall dotted with various kinds of channels that pump molecules ‘uphill’ (from low concentration to high concentration, or the reverse). Without that, all living things would die. Many cells burn half their energy transferring molecules across their barrier.
But once again, that pest Aristotle interrupts to point out that all that seems far too unlikely to occur at random. For example, such a network’s existence depends on water pressure (that is, energy levels). If the water only trickles in, too few parts will bump into each other per second for their reactions to make enough copies of themselves. But if the water jets in too strongly, many parts will bump into each other per second, but the fast-moving water will cause so many collisions that the resulting masses will break apart too fast for many copies of them to persist. The network’s existence also depends on resource availability and waste removal. If the bag doesn’t start with enough different kinds of parts, or if enough different kinds of parts don’t flow in per second, or if enough of the parts that could clog needed reactions don’t flow out per second, the whole network might never start; or if it does, it might quickly collapse.
He’s right, of course. However, we don’t have to assume that all that had to have happened just the right way just the one time in just one bag. If we could somehow make many bags, each with many sets of initial parts and various water temperatures and pressures, it seems at least possible to imagine making a real cell if we could also shrink each bag and its contents about a billionfold. Then we would just need to pour more copies of the original parts into the bags, and keep pouring for, oh, half a billion years or so. Each bag would then be about the size of a real cell. Its parts would be about the size of average molecules. At that size, they would be surrounded by water molecules and jammed into the bag cheek-by-jowl. They would also be bopping into each other at about a billion times a second, jittering around at about 500 million times a second, rotating at about a million times a second, catalyzing each other at about a thousand times a second, and what we would have in the bag is... something like life?
That’s a tall stack of ifs; but if it could happen, then the bag of reactions would now have phase changed. Its bag would now be its ‘cell-wall,’ giving it an inside and an outside. Also, the parts that it takes in and uses in some of its reactions but which none of its reactions make would be, in a sense, ‘food.’ The network needs them but can’t make them. Parts that some of its reactions make but that none of its reactions need would be, in a sense, ‘waste.’ The network makes them but can’t use them. Thus, the bag of reactions would now be, in some sense, ‘eating’ and ‘excreting.’ As long as it’s bathed in parts and energy, its density and diversity may well help it keep doing so. If so, the whole would be more than the sum of its parts.
Such a hypothetical thing seems to be nearing Aristotle’s ‘entelechy,’ at least in the sense of ‘working to remain oneself,’ since, given the right circumstances, it could arrange matter and energy to persist, and perhaps even accidentally multiply (although not reproduce—that would require information preservation). But whereas with that name he posited some unseen purpose inside every living thing, we might be creeping a little closer to a possible explanation for how such a thing might actually work.
Further, that’s not only something that might have happened to molecules on this planet billions of years ago. Simpler versions of it may have happened to us, too. Thus, some innovators in the United States in the 1800s, building on the work of others in France and Britain (who had built on earlier work going back millennia), stumbled upon mass production. The production system that they built up over time could go on to make lots of things, and the things that it needed to make those things were themselves makeable inside the system. In a sense, it was in a bag, and it could make itself, and its own bag. It could do that because it could make its own machines, so it was a machine that could remake itself. That made it attractive (vast amounts of land had earlier made the country attractive for other reasons), and that attractiveness (not to mention new military power...) made various resources ‘flow uphill’ into the country. All countries that turned that particular corner became autopoietic, and for them, various resources then flowed uphill.
Frothy Bubbles
Our reanimated Aristotle might well accept various recursive properties, but now he may object that the first cells must have had still more structure than those properties might explain. Sure, he may concede, for a reaction network to be self-maintaining it must at least have catalytic closure (that is, it must be synergetic), or else it would soon cease to exist. It must also have operational closure, or else it would lose ‘trade power’ against its surroundings, and thus would soon starve. It must also have a skin, or else its parts would soon lose density. It must also use some of its byproducts to maintain that skin, and that skin must have pumps to fetch parts (from which it also extracts energy) and extrude waste. That is, it must be autopoietic. If not, it couldn’t keep itself diverse. And only with diversity and density could it continue to retain catalytic and operational closure, and thus continue to be self-maintaining.
But, he may then complain, the cells of the living things that he can see with his new microscope have far more structure than just their skins alone. Those cells aren’t just bags holding a jumble of reactions. Were that so, their reactions might interfere with each other. And if they did, the whole thing could fall apart, so they couldn’t build on each other to make olive trees and bees and us.
So, Aristotle may argue, to be truly ‘life-like,’ a reaction network must also be organized in space. And it must maintain that spatial order, just as it must maintain its own skin—or else we’re back to his unseen somethings that organize everything. Thus, for a self-maintaining network to one day become something like today’s highly structured life, it must gain, and maintain, some kind of at least minimal internal structure. That is, its bag must not only have parts, it must arrange where those parts are in relation to each other so that they work together to persist themselves.
Then, soon after he mentions space, he’s going to think about time. If a cell has to have a geography, transport times are going to matter too. Parts in transit from one place to another need to move, or be moved, faster than they decay or are consumed, or else there’s no point moving them (or even making them). Also, reactions that make copies of a part need to work at least as fast as the reactions that need copies of that part—plus the time to transport those copies. So, he may argue, surely there must be some unseen ruler or guiding hand or some such thing that specifies not only where everything is supposed to go but also when everything is supposed to happen. Further, for that to happen there must be lots of infrastructure to ensure that everything is in, or gets to, the right place at the right time.
In short, Aristotle may now argue that in ‘truly living’ things, perhaps even the first thing that we today would be willing to call ‘living,’ not just matter and energy, but also space and time must all be in delicate balance. That is, he may now move his need from some guiding hand, some built-in purpose, back up from the first cell’s molecules to that cell’s structure. So what, if not some unseen something—to please him, call it a soul—keeps the balance?
Well, we don’t know, nor do we know if that had to be the case for the first true cell. But if space and time had to matter inside that cell, that probably couldn’t work without some sort of transport inside the cell. How might that have happened?
Take one particular group of proteins known today, the kinesins. They shuttle parts around inside a cell by latching onto bags of them then zipping along cables inside the cell. That can rush a part across a cell about 300 billion times faster than it otherwise would have moved by random drift. One such kinesin is made of 5,743 atoms. It works something like a blind swimmer carrying a backpack full of cargo up a ship’s anchor cable. That swimmer runs out of steam after moving from one rung of the cable to the next. However, packets of sandwiches (ATP molecules) are bopping around in the murky sea, and the diver gains the energy to ratchet along one more rung of the ship’s cable whenever a sandwich happens to bump into it. So, if there happen to be lots of sandwiches, it blindly inches along the ship’s anchor cable, carrying cargo from place to place. The cell powers those transporters by producing lots of sandwiches and just flooding its internal sea with them.
On describing such a bizarre, but highly intricate, precision piece of atomic engineering to our revitalized Aristotle, he will surely say that it must have been designed for precisely that purpose. It’s too necessary to the cell’s persistence, too well-fitting with everything else in the cell, and too efficient at what it does to have happened by chance. He would be right, too. No such thing could just pop into existence—and it surely has a ‘purpose.’ But still he would be making a mistake.
He’s assuming that the cosmos is both perfect and unchanging. For him, everything that exists now has always existed. So he’s balking because he’s trying to imagine how a cell could simply pop into existence with its cables and kinesins and such, intact.
But if we’re already past the point in Earth’s history where information could be preserved in some way (not necessarily via today’s genetic machinery, but perhaps some proto-form that’s now lost), then we might rely on an evolutionary explanation. The parts of such a transporter system needn’t all have come to exist at once. Nor, once they existed, need they have fit together as intricately as they do today.
As far as we today can tell, the cell’s free-floating sandwiches (ATP) came first, over three and a half billion years ago. They’re handy power cells for most reactions, and they occur in all known cells (so perhaps those may be descendants of something used in early proton pumps across cell membranes; that may be how protocells gained energy from the energy flux they existed in). The cables may have come next, well over two billion years ago, since variants of them exist in many cells. Nor need they have arisen to allow faster transport, for in many cells they both help maintain cell structure and help support cell division (so perhaps those may be descendants of some rope-like thing that helped some of the earliest cells divide, or perhaps it at first had no use at all). Then, perhaps, came some simple motor that relied on ATP, then, maybe, some simple gripper that attached to and detached from the cables. Then, perhaps, came linkage of the motor and the gripper, then a backpack for cargo storage, then linkage of the backpack with the grippers, and so on.
Each of those parts may have first had other uses in the cell—or else their precursors may not have arisen at all. If so, then once they all existed in a cell together, they would all have been linked into one reaction network, so they may have been under pressure to change jointly over time as threats to the cell’s persistence changed. If specialized descendants of them then slowly joined together into the first crude transporter, that would have given the cell slightly faster cargo transport, which, likely, gave such cells an edge over competing cells. All the cells that didn’t manage to change in that way may have either died out or split off into different kinds of cells. Thus, the parts make up the whole, but perhaps the whole also selects the parts.
If so, Aristotle, by looking at living things around us today and assuming that they must always have been much as they are now, would be looking through the wrong end of a four-billion-year-long telescope.
But he does still have a point, for while we today are beginning to work out bits and pieces about how various cellular machines may have come about, we still can’t yet answer his newest questions because we don’t have any clear idea how a shapeless network might have come to have spatial structure. However, if something that starts as one bag could (somehow!) gain smaller bags inside it, specialization could become rampant, production could become localized, and inner barriers could rise up everywhere.
If an autopoietic (self-maintaining) network could (somehow) gain persistent internal parts other than its outermost bag, then it might become stigmergic (‘self-building’). Reactions between its (passive) structure and its (active) reactions then become possible. How, though, might that have happened?
Well, we don’t know.
But in 2010 we built our first life-form in the lab; however, like Frankenstein’s monster, we had to make it out of already made parts. We don’t yet know what all the parts do. Then in 2013 we built our first crude version of a ribosome, the single most complex part of a cell, from scratch. So it may be only a matter of decades before we build a complete cell entirely from scratch.
One day we might live in a world where someone may say to you: “Today I wrote a new fugu. Want to see?” And they won’t mean a new piece of music. Nor will they have mispronounced a word. They will mean a new fish.
However, that’s probably far in our future. Until we can watch a completely synthetic cell work when we do X, and not work when we do Y, we’re going by guess and by gosh. But after many decades of work and thousands of research papers we’re beginning to guess at how a bag might come to contain bags inside it. Perhaps if those inner bags could somehow become useful to the whole bag (as mitochondria or chloroplasts are for today’s cells, for example) there would then be pressure for things like transporters and such to come to exist so that the whole thing would mesh together stigmergically and thus become more likely to persist as a unit.
The above process has question marks scribbled all over it, but if new reactions could develop in such a compartmentalized reaction network, then it might begin to change in an ecogenetic (‘self-assembling’) way, much as food webs change when new life-forms invade. Its structures could then help constrain its reactions, and its reactions could then help maintain its structures even as new reactions develop within it. In time, such new reactions might even help their network begin to ‘sense’ food, ‘engulf’ food, convert some food into more storable form (like fats). Yet others may help it ‘void’ waste, move around, attack other reaction networks.... After all, stigmergy is just the special case of ecogenesis where there’s only one (self-)helper reaction in a network.
Without stigmergy and ecogenesis further changes seem unlikely. But if a network could (somehow) add structure to itself, and thus become stigmergic, then ecogenetic, it seems increasingly hard to deny that it wouldn’t then be, in some strong sense, alive.
However, Aristotle could still continue to argue. While we might one day show that the first true cell—in today’s meaning of the word—might indeed have self-assembled billions of years ago, today’s cells don’t do that. They build themselves on top of, and with parts from, their parent cell(s). Further, most of today’s cells can do more than that. They can repair themselves when damaged, and they can copy themselves. They do so with the aid of a marvelous structure that acts as a recipe book of the cell itself. A cell can use that recipe book (its set of genes) to repair itself. Plus, it can write copies of that recipe book into its copies, so in that sense it can write itself. The genes in a cell replicate, but they don’t reproduce—cells do; and for that to happen, a cell needs more than a recipe book; it also needs many intricate parts, which need to be highly ordered spatially. We only have vague ideas about how all that might happen.
Thus, Aristotle might point out, a cell today isn’t merely self-building (stigmergic) and self-maintaining (autopoietic); it’s also both ‘self-repairing’ and ‘self-writing.’ Plus, to be self-repairing, it must first (as far as we today know) be ‘self-describing.’ That is, it must (somehow!) become like a sentence that contains a description of itself. And since it’s also self-writing (that is, it can copy itself), it’s thus also a sentence that can write itself. How, though, could such a sentence complexify itself enough to become self-describing? Then, how could it compare that description with its current state so that it could repair itself? Then, how could it write itself? Then, how could it assemble many copies of itself into organs, systems, whole bodies? There are so many questions.
One day we might devise some plausible explanation of the phase changes from non-living to self-helping (autocatalytic) to jointly self-helping (synergetic) to self-maintaining (autopoietic) to self-building (stigmergic) to self-assembling (ecogenetic) to (the big three) self-describing, self-repairing, and self-writing. If we do, we could finally say that we can explain all of self-generation. But life is enormously complex, so that time may be far into our future. For all that time, and even beyond, we would always have Aristotle’s explanation. And it’s far simpler.
Swarm
So far, conversation with replicant Aristotle about what a living thing is has mostly focused on cells, but given examples like Portuguese Man o’ War—which aren’t animals but colonial life-forms—or slime molds—which aren’t fungi but colonial life-forms—or seaweed—which aren’t plants but various assemblages of algae—or lichens—which aren’t fungi but symbiotic messes of fungi and algae—looking at life in terms of persistence rather than behavior or substance might make sense for larger things, too.
Consider termite species that build large mounds, some 17 feet or more high. Normally, such a mound looks dead, but on rare evenings, especially after a rainstorm, it might boil with activity. Thousands of winged termites gush from it, like water from a pricked firehose. Flitting here and there, they seem desperate to learn how to fly in minutes—which is wise, because toads, lizards, and snakes will soon hop, skip, and slither in for the free buffet dinner. So the fliers barrel off as soon as they can, scattering like bomb shrapnel.
When a female lands she sheds her wings—wings she took months to grow—wings she never used before and will never use again. Then she balances on her forelegs and raises her hindquarters. Frozen in place, her scent calls males to her. After a time, one may come buzzing along. The two of them then scurry about until she finds some place that suits her in the dark, rain-moist earth. There they burrow, hiding from the birds that will come in the morning. If she lives, she’ll become a queen.
Years later, instead of one mound, there might be two. Inside that second mound would be a city, with as many as two million termites. Some repel invaders, other farm fungus on chips of moistened leaves, grass, or wood. Some nurse the eggs and young, others fetch water. Some scout, others forage. Some build, others explore. Almost all are neuter, wingless, and blind. All are offspring of the queen. But her one Cinderella night of flying on gossamer wings followed by hot sex is long gone. She’s now a hundred times bigger—so bloated that she can’t move—just a glistening sac, stuffed with new ovaries—with her wings gone, but with her tiny waving legs and antennae still attached. She’s now little more than a biological machine gun, pumping out an egg every three seconds—30,000 a day. Then one rain-moist evening, after the birds have nested but before the owls and bats hunt, that new nest will churn with activity. Sighted and sexed fliers with newly minted wings will boil out then buzz off into the night.
Were we to ask our recrudescent Aristotle how that whole cycle happens, he would probably say it’s obvious: the queen orders each step. He’s likely to say that because, of bees, he thinks their ‘king’ tells them what to do. (He thinks their queen is a king because it has a stinger, and, for him, no female carried weapons. He’s still puzzled, though, because he’s never seen any bees having sex, so, for him, they’re neither male nor female; and the ‘king’ takes care of the young—and, for him, only females did that.) So for him, the queen rules.
But centuries before he was born, and to the southeast, another careful observer, looking at ants, saw something different, writing: “Go to the ant, thou sluggard; consider her ways, and be wise: which having no guide, overseer, or ruler, provideth her meat in the summer, and gathereth her food in the harvest.”
That’s true for termites, too, for what matters isn’t the queen, or any other single termite, but the colony as a whole. Even killing the queen might not trouble the colony much, since in some species the colony might just grow a new queen. The queen’s brain, about the size of a pinhead, is no bigger than that of any other termite, and has only has about a million or so neurons. That’s probably too few to organize the actions of millions of termites. Even if it weren’t, she’s wedged into a cavity just big enough for her newly bloated body. Even if she could escape it, the tunnels are a hundred times too small for her. Even if they weren’t, she might be entombed perhaps a dozen feet or more below the nest’s top. And while her scents can trigger some things—like suppressing reproduction or triggering a major change—they can’t dictate everything, just as ovaries in human females trigger monthly changes but don’t determine everything. Her controlling every action in the nest would be like one of us shouting orders at the bottom of a skyscraper two miles tall and five miles wide.
So Aristotle is wrong. We don’t have to assume a big-brained termite, nor even a politburo of small-brained termites. Nobody is in charge.
However the colony does have a brain, of a sort, but it isn’t a lump of flesh. It isn’t even just all the little termites brains put together. It’s spread out across many more parts, including the termites’ genes, and their modifications to their clay nest, and the scent trails they leave behind as they work. All of those parts affect their actions.
Those three sets of brain parts extend the colony’s ‘memories’ of what’s important (besides any memories that the termites themselves may carry in their tiny brains). The genes ‘remember’ its deep past, perhaps millions of years ago. The nest’s structure ‘remembers’ its historical past, perhaps up to a decade or two before. The scent trails ‘remember’ its recent past, perhaps a few days before.
We aren’t (yet?) used to functioning while parts of our brain are scattered all over the house and yard, but the colony’s arrangement is something like our brain’s long-term, short-term, and working memory.
A termite, by offloading its recent memory, can talk with—and thus work with—other termites (or even with itself), in ways not otherwise possible given its small brain. That then leads to stigmergy. For instance, if a termite follows a scent trail and finds food, it drops scent on the trail on its way back. That encourages other termites, or it itself, to follow the same trail. (That’s also an autocatalytic reaction, but laid out in space as well as time). So rather than having to remember what it’s doing for more than a few minutes at a time, it gets the world to remember what it’s doing by writing its actions into space, thus recording its actions in spacetime. We do the same, except that our brains are so much larger that we can keep more of that space inside our heads.
While to Aristotle the colony might appear to act as if it’s governed by one ruler, it has no single decider. There’s no ruler—or rather, it has millions of rulers. It’s just a mass of termites, each reacting to each other’s actions and changes in the world around them. A cell is similar. A cell is a complex machine with thousands of moving parts, each of which may be made of thousands of pieces.
Each of the colony’s termites is like a protein in a cell. However, unlike most proteins in a cell, many termites in a colony can change roles as conditions change. A cell can do that only by changing the numbers of each kind of protein. So, thinking of a protein like a single-function robot, a termite is like a multi-function robot. It’s like a robot that can slot in different tools at different times, and, like changing protein production in a cell, it does so depending only on local data, not some global decider.
Like a cell, the colony’s overall behavior is made up of many small actions triggered by other small actions, which are triggered by yet other small actions. Like a cell, some of the colony’s termites build stuff up, others break stuff down, some fetch stuff, others drag stuff away, many (all?) act on each other via scent and touch, while just one (the queen) replaces worn parts. Like a cell, that gives the colony control over energy buildup, storage, and release to thus shape matter and maintain that shaped matter, which then helps it continue to shape matter and control energy, and thus persist.
Like a cell, for the colony to persist it needs energy and parts. It needs parts to capture energy from some energy flux (in its case, fungus breaking down cellulose into sugars). And it needs more parts to replace and repair parts lost doing that. To make the first work, it needs catalytic closure; and to make the second work, it needs operational closure. But unlike a cell, it’s not inside a bag. Its active parts keep themselves close enough together to continue keeping themselves close enough together. (Those that don’t, die.) Its ancestral line somehow figured out how to do that, and put the trick into its genes around 31 million years ago.
Like a cell, the colony divides all its labor—including reproductive labor, which the queen does all of—and it ‘decides’ to change its tasks, and perhaps change which termites do them, or how many termites do them, as threats to its persistence change. Depending on the threat (or the need), it has fast responses, slow responses, and very slow responses, and a cell is similar. However, how cells and colonies do things differ. For example, a cell quickly changes what it does by changing its numbers of robots, whereas a colony quickly changes what it does by changing which robots its robots are. Thus, like a cell, a colony is a self-persistent reaction network. It acts solely so as to keep acting.
When a cell (or colony) is ready to copy itself it ‘asks’ different things of its molecules (or termites) than it does when it’s not ready to copy itself. For instance, those sexed, sighted fliers that fling themselves out of their nest every few years usually are rare in the nest. Eyes and wings and sex organs make no sense in narrow, pitch-black tunnels where only the queen makes eggs. So the colony usually doesn’t build many of them—until it decides to try to copy itself. Then it prints them on demand—like parts off an assembly line—but only when it can afford to make so many that at least one male-female pair might survive the flight and copy the colony. Thus, those female fliers aren’t some sort of daredevil insect Amelia Earharts, swathed in goggles and scarves. They’re more like dandelion seeds.
Like a cell, the colony has many disposable interacting parts, so its actions are deformable over time. So it may be able to adjust to changes in the world around it in ways that a single termite (or a single molecule in a cell) cannot. Even were each of its termites to always behave exactly the same way, since it consists of a variety of them, merely by selecting the numbers of each variety that exist at any time it might be making a ‘memory’ of that moment. So how it might react in future to the same threat may vary. (Note however that thinking that way may lead to strange ideas: If those ‘memories’ cause it to act in some consistent fashion over long periods, maybe we might even say that it would be developing a ‘persona.’ Then, were we to accept that, we might go on to say that the purported persona is, in some sense, ‘thinking,’ and so on. After all, if we could take a molecule’s eye view inside our own brain, would it be all that different?)
Like a cell, the colony persists only if all its parts, both passive and active, interact fast enough to meet threats to its persistence. Like a cell, it has to ‘decide’ which stimulus to ‘pay attention to,’ and what action to ‘select’ in response to each stimulus. Stimulus. Response. Action. Reaction.
Like a cell, at any moment, the colony may have many stimuli, and it may have many actions as options. It thus needs some sort of stimulus-action map to match one with the other. And a stimulus may be external or internal, and an action may itself be stimulus for another action. Also, that call-and-response map needn’t be static, it may be dynamic—it may change over time. Further, no single termite, not even the queen, need be aware of all its colony’s possible threats, needs, and options, nor all its colony’s possible reactions to those, nor all its colony’s potential changes to its map, but, in some sense, the colony must be—or else it wouldn’t persist. Thus the colony is a network whose structure gives it roughly the same effect as a gigantic computer program with a whole lot of rules (including any rules that change other rules), sort of like: ‘IF this, THEN that, ELSE the other,’ but all jumbled together, where the ‘this’ and ‘that’ and ‘the other’ may melt and meld and reform and otherwise change over time.
That’s what keeps the colony going. If the colony (or cell) has a ‘soul,’ that’s it.
Ideally, if we knew that stimulus-action map, we could build a model of a colony and see how it behaves over time, but we don’t, so we can’t. At least, not yet.
Like a cell, a colony’s behavior is hard for us to predict because it’s hard to detect precisely and also to model mathematically. That’s because, until recently, most of the networks we modeled mathematically had only a few parts, each of a fixed type, and links between those parts were fixed. However, these networks have many parts, and their types, numbers, and even linkages, may change over time. Further, in a colony (or cell), it isn’t enough for many parts to work together; when and where they do so can also be critical. For such large and fluid networks, saying anything with precision is hard because detecting what’s where when is hard. The math is hard, too, because small changes might trigger rapid and large-scale rewiring of the network. So, in effect, such a network isn’t one network, but many, all smooshing together dynamically from one to another. Its dynamics are complex. We still lack enough hard data and mathematical tools.
Thus, a termite is an animal, but a termite colony isn’t merely a set of animals—nor is it the nest those animals build, nor the scent trails those animals leave—any more than a cell is just a set of molecules. It’s more like a tree on a million legs—or a brain with a million noses. It’s a composite thing—not a plant, but not an animal, either. Like an animal or a plant, it keeps itself going because its parts mesh together to make and maintain more of its parts fast enough for enough of them to keep going in the face of varied threats to its persistence. And when it can, it tries to copy itself.
Finally, while a colony is made of parts, not all of them are alive. And while we can touch each of its parts, its essential part—just like Aristotle’s crab-soul—isn’t touchable. That’s the non-physical network itself. The thing that perpetuates itself the longest isn’t so much the parts, but their pattern of interactions. That isn’t any particular termite—not even the queen—nor any particular scent trail, nor any particular chunk of dirt. It might survive the loss of any of those—if the loss happens slowly enough for it to recover in time. It, plus all the matter it animates to sip from the energy flux it’s in, is a self-maintaining patterned distortion of matter and energy moving through spacetime. That’s a time-traveling termite-thing. Call it a termite swarm.
That swarm has many of the signs of life that Aristotle first listed over two millennia ago. It doesn’t merely resist dissolution for a while, the way a vortex (a tornado, hurricane, whirlpool, waterspout, and so on) does; it acts to persist itself. It eats, grows, and senses and reacts to changing conditions. And it reproduces. And while its active parts are tiny, a large colony breathes about as much as a cow does; and in an average year, it moves over 500 pounds of soil, several tons of water, and shapes the equivalent of a complex city. Its queen isn’t an ovary; she’s more like a stem cell—something capable of making any number of any other kind of ‘cell’ in the colony. It also has external additions for a lung (its mound), a stomach (its fungus farm), and a brain. Not to mention that it farms. But it isn’t a single gooey mass of cells. Nor do all its parts have genes. And while it has some parts that are separately alive, it also has parts that aren’t alive at all. So is it alive or not?
Posing that question to our resuscitated Aristotle might make him squirm, since even today’s biologists struggle with it. But then, given life’s huge variety, biologist don’t even agree about the meaning of the word ‘organism.’ Some biologists call termite (and ant, bee, wasp, and other) swarms ‘superorganisms.’ Many biologists, though, don’t use that word because it suggests that what it describes are organisms, and are thus alive, but that’s the question—are they alive? The word ‘swarm’ may not presume quite as much. The idea of a swarm is that its parts, unlike the parts of a typical life-form, needn’t all have the same genes, needn’t each be alive, needn’t all be touching, and so forth.
It’s hard to fit such networks into our current theory of evolution, because that theory mostly doesn’t apply to networks of parts but the parts themselves. Even that is a problem, for evolution today is mostly still about things that copy themselves, so what do we make of a network part that can’t copy itself? For that reason, Darwin struggled with termites and similar colonial insects. Partly because of them, he held off publishing his Origin of Species for almost 20 years. To him, they were: “one special difficulty, which at first appeared to me insuperable, and actually fatal to my whole theory.... sterile females.... cannot propagate their kind.... this is by far the most serious special difficulty which my theory has encountered.” It took a whole century for biologists to devise even a partial solution to that problem. Plus, that only worked for colonial insects that are near clones of each other (like ants, and some bees and wasps). That’s not true of termites. So it’s still an open question in biology today.
We could put all such weirdo things in one bag were we to change our definition of life-form, expanding it from our old Aristotelian one of behavior (what it does), and even our new Darwinian one of substance (what it’s made of), so that the defining characteristic of all living things becomes structure (does it do work to help itself persist?).
Is that crazy? Well, maybe. But imagine a relay race where the runners pass batons for as long as they can. The runners may die, and even the batons may wear out, but as long as they all get replaced before that happens, what persists isn’t the runners, or the batons, but the race. The point isn’t how the race is run, or which runners are presently running, but that the race is running.
For instance, we think of an adult mayfly as alive, but it’s also part of a pattern—a cycle that begins with an egg. That egg can neither eat nor move nor reproduce, and some of its parts (its shell) aren’t themselves alive. It then becomes a larva, which eats and grows but can’t reproduce. That larva then becomes an adult, which can neither eat nor grow but can move and reproduce. That then leads to another egg. The point is that we can predict something of what we will see in the future because what we identify as one physical thing—the mayfly—is an instance of another, but non-physical, thing—the mayfly cycle—a pattern moving through spacetime. If that’s what’s important, then maybe it’s sensible to generalize the concept of life to networks that self-persist?
But if we do, then what are we? Like termites, we’re all animals, each separately alive, but what is a planetful of us plus all our tools and all the things that we’ve built with them over the millennia? All of us together isn’t the same as each of us separately. The thing that persists isn’t any one of us—we all die—but the reaction network that we and our tools and their products form. Just as termites build, what is to them, a giant nest out of clay and spit and dung, so are we building a planetary nest with our tools. Just as their nest shapes them, so does our nest shape us. We and our tools—physical and institutional and mental—are locked together into a giant reaction network, a network that self-persists. It hasn’t reproduced (yet?) but it’s a time-traveling human-thing—a human swarm.
Life is a Verb
In the swarm-physics view, a ‘living thing’ needn’t necessarily be a single touchable thing. It might be a network of separate but linked parts. Those parts needn’t always be in contact, nor need any of them persist for long, nor need all of them, or any of them, even necessarily be themselves ‘alive.’ As long as they interact fast enough so as to recreate enough copies of themselves so that they stay networked, their network persists, and thus is ‘alive.’
In this, admittedly strange, view, neither an isolated virus nor a fire nor a vortex is alive—or if they are, they needn’t be alive for long, since they don’t self-persist. However, a cell, a kitten, a Portuguese Man o’ War, a lichen, a virus in a host cell, even a termite swarm, all work much the same way. They’re like self-exciting vortices—or, self-running relay races. They exist from moment to moment solely because their parts keep remaking each other, which keeps remaking them.
To do so, such a reaction network must make many of its own catalysts—most of them are too intricate and delicate to come to exist in many copies otherwise. But to do that, it needs a constant supply of matter and energy. If its supply is interrupted for too long, then even if given a fresh supply later, its parts may not be able to reestablish all their links; so their copies decay, and they all depend on each other. It’s also sensitive to its amounts of ‘food’ and energy. Without enough food, it ‘starves.’ With too much food, it ‘chokes.’ Without enough energy, it ‘freezes.’ With too much energy, it ‘burns.’ It’s thus constantly balancing on the edge of a knife. It can ‘die’ in many ways.
To persist, it needs many delicately balanced reactions, which need many parts. The ones we today know of need at least a few thousand different parts reacting together in at least a few thousand reactions. Below that threshold, networks may not be diverse enough to be fully self-helping—at least, not with the chemistry or entomology that we’re familiar with at low pressures and temperatures here on Earth. Further, each network part needs to exist in many thousands of copies. Thus the network’s parts must be both many and diverse. Yet they must also be dense. If they aren’t ‘near’ each other, their reactions can’t ‘trade’ ‘goods’ before those goods die out, or before the reactions that depend on them die out. Were that to happen, reactions wouldn’t be able to aid each other. So the reaction network must be both diverse and dense.
No single reaction in such a dense and diverse reaction network can persist alone. Each will die out unless it’s ‘near’ some particular subset of the others. That subset acts as a general ‘factory’ that makes the reaction’s parts. Further, for each such factory, there must be yet more factories to make their parts, and so on. So every reaction depends on the whole network, and the whole network depends on every reaction. Thus, we can’t break up the network into independent pieces. It’s spatially non-linear.
All reactions in such a spatially non-linear network are synergetically linked. So whatever affects one, affects all. If the conditions of any reaction changes, that change will propagate to all others—after some lag dependent on reaction speed and transport linkage. For example, if, for whatever reason, the number of copies of some catalyst rises, then all the reactions that it catalyzes will speed up. They then make more parts, which may catalyze yet other reactions. So those may speed up, and so forth. The synergetic cycle then closes back on the first reaction. So not only is the structure of the reaction network non-linear, each reaction in such a network also grows non-linearly. It’s temporally non-linear.
Such a network is thus inherently dynamic. It can never just be—like a diamond or a stick of chewing gum—it’s always in the process of becoming. It’s thus able to react to, and perhaps even compensate for, changes in its surroundings.
However, at constant temperature and pressure, a catalyzed reaction can speed up in proportion to the amount of both its catalyst and its reactants (that is, the parts that the reaction’s catalyst acts on). Reactants get changed into products as the reaction proceeds, but the catalyst itself remains unchanged. Further, both the catalyst and the reactants are produced by other reactions, and all reactions are linked. So the amount of any particular reaction’s catalyst goes up as the overall reaction rate goes up. So its growth rate can’t be linear. It may not even be merely exponential. It can be hyperbolic. (That is, it might grow as the square of the amount of catalyst available to it, and thus is doubly exponential—its exponent is itself exponential.) Thus, once beyond a certain threshold, the reaction network might take off, burning through its reactants. Once started, it can’t stop until it’s ‘eaten’ all of its scarcest resource—then it’s ‘hungry’ for more. So it’s ‘greedy.’ (‘Shortsighted’ works too.)
However, no such greedy network can speed up beyond a limiting reaction rate because all its reactions are synergetically linked. So whichever reaction that needs the scarcest ‘food’ fixes the maximum reaction rate of the whole network. During network speed up, all linked reactions will—after various lags—automatically adjust to that speed. Once things settle down, the reactions can go no faster (and no slower) than that overall reaction rate. Such a network can thus adjust to some change but then resists further change. It also can only exist at a certain heartbeat. It can’t go faster, nor can it go slower, than a certain rate inherent in its structure. It thus persists only when it’s ‘conservative.’
Also, because the parts of such conservative networks will decay or be consumed at various rates, their resupply rates must match or exceed the slowest transport time between the network’s parts relative to the decay rates of the parts being transported. For a synergetic network to persist, every part of it must persist; and for that to happen for each part, the network must have some subset resupplying that part (or making new copies of it). Also, all the parts of that resupplier subset must themselves have subsets resupplying them, and so on. That then limits how ‘far away’ any part can be from its resupply subset. In turn, that limits the size of the network for a given set of die out rates plus transport times of its parts. That applies whether the network is contained in a physical skin or not. Any portion of the network that drifts too far from its resupply subset must die off—as is true, for example, of termites that wander too far from their nest. In short: the faster a network’s parts die out, the faster the network must run, or the denser it must be, or both.
Further, the network’s parts must work together so that they, or copies of them, jointly persist into the future. Or more precisely: they don’t persist if they happen not to work together. So while each part must be helpful, it needn’t be intentionally helpful—or intentional in any way at all. So, contrary to Aristotle’s view, nothing in the network need be purposeful, goal-directed, or forward-looking. No part nor reaction need have any built-in tendency to help others persist. The network will still be composed of such parts, because those networks that don’t will cease to persist. Thus, all persisting reactions of every synergetic network are, and must be, tit-for-some-tat.
In sum, a self-maintaining reaction network could potentially be made of separated yet interdependent parts. Such parts might be living or non-living. But their network must be dense and diverse. However, the ‘distances’ between those parts depend on their rates of decay. (That is, the shorter-lived the network’s parts are, the closer-lived or faster-transported they need to be, like perhaps in a cell. A network made of longer-lived or faster-transported parts might be larger and more widely spread out, like perhaps a termite swarm, or maybe even a planetary human swarm, if such a thing can exist.) To persist, the reactions that those parts belong to need constant flows of matter and energy, and they’re sensitive to the amounts of such flows. Further, not all of the matter that the network transforms is makeable by or usable within the network. Such networks must thus ingest and excrete. They can also die out in many ways. They also have a boundary of some sort limiting how far away any part can be from other parts yet still be supported by the whole. They’re non-linear. They’re unstable. They’re cooperative. They’re conservative, but also greedy. And they at least seem to be purposive—at least as perceived from the outside—if only in that their purpose seems to be to try to persist.
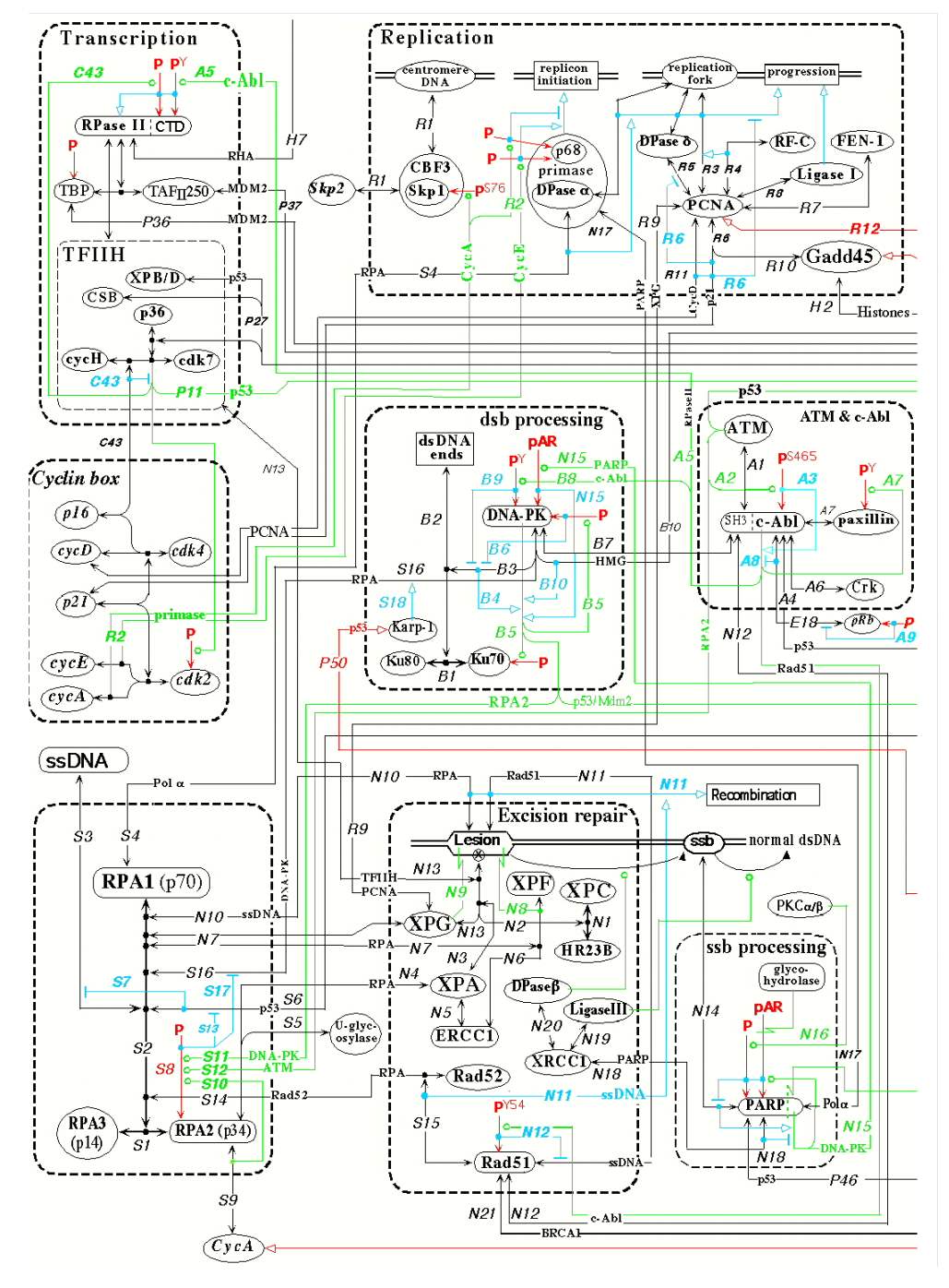
A small part of a single cell’s reaction network (From K. W. Kohn, 1999. Used by permission.)
In this view, a living thing isn’t its parts. It’s a special kind of pattern inscribed in the reaction-network dependencies between its parts. It’s matter and energy informed so as to direct and control matter and energy. That’s what lets it persist. It’s a self-writing sentence, which once written into the cosmos tries to keep rewriting itself into the cosmos to thus keep itself there.
Our bodies, too, are patterns that persist themselves. We aren’t our goo. We aren’t our genes, our brains, our sex organs, nor any other of our parts. We’re sets of constantly changing links between those parts, and those parts are constantly falling away and being replaced by copies. Each of our bodies is a reaction network of perhaps ten trillion human cells mixed in with around 10 times as many microbial cells: bacteria, yeasts, fungi, viruses, and others, which add up to around five extra pounds. Every day around ten billion of our cells die or commit suicide, but new ones replace them fast enough so that the network as a whole keeps going, usually for at least a few decades. Further, the average such cell might have perhaps 50 million proteins, any copy of which might be consumed, damaged, or destroyed at any moment. But that usually doesn’t matter because those proteins maintain their network by bopping into each other at roughly a billion times a second. Each of us is thus the same as a set of organs as a rainbow is the same as the set of water droplets that refract its colors.
Thus, in the swarm-physics view, we aren’t just our bodies; we may be more like waves in water. In this view, we’re resonances, recursive time-traveling network effects scrawled into the cosmos. Our swarm seems to be similar. Both we and our swarm are like self-writing patterned distortions moving through spacetime—sentences that exist only for as long as they can keep writing themselves into the cosmos. In this view, life isn’t a noun. It’s a verb.
Beauty Is Difficult
Aristotle began his Physics 2,300 years ago by asking why the cosmos was the way it was. Why did things, especially living things, have the forms and behaviors they have? Why was there order and not chaos? Most of us who grew up in his time, and for millennia before, already had an answer: divine intent. That explains everything because it explains anything at all, and nobody can disprove it. But by his time a few of us had a different answer: sheer chance. That also explains everything, and for much the same reasons. However, Aristotle must have disliked both answers, for he kept searching, as a few others had in the centuries before him. Maybe he felt that an explanation with no limits explained nothing at all, since it explained everything exactly the same way. Perhaps that’s why he thought that the order he saw all around him was the result of everything—children, trees, water, rocks, stars—having built-in purposes.
He may have come by his idea of purpose in the cosmos by analogy with things we make. He could see that a carpenter crafting a table and a sculptor chiseling a statue both plan their work. Their mental pictures of what they want then guide them to the things they build. The same, he thought, must go for everything—crabs, oysters, olive trees, planets—not just the stuff we make. Everything was either made by us or by the cosmos. For him, there was nothing in-between. Thus, everything had purpose, and it was either inborn or inbuilt, never both.
But he missed something. What about complex networks, like cities and economies and such? Where did their order come from? If they’re to have purpose, is it inborn or inbuilt?
He didn’t think of them the way he would have thought of bee hives (or, had he given them any thought: ant hills, or termite mounds) because, he thought those must have rulers, and he knew that rulers didn’t explain everything about our cities or economies. We know that because in his Politics he said that cities somehow share in both inbuilt and inborn purpose—thus contradicting his otherwise strict separation of origin of purpose in all his other books. A city, he said, had a ruler or rulers, but it also had a politeia (a ‘constitution’ or ‘regime’ or ‘way of life’ or ‘mode of rule’). Nor was that something we wrote down and all agreed to. It was a non-physical principle—the soul of the city.
Why did he waffle? When it came to cities he probably had to because he could see that we didn’t make such networks; they grew. But the cosmos didn’t make them either; they grew out of parts we made. So cities were neither like sculptures nor crabs. We made them, but we didn’t plan them. Oh, like the carpenter and the sculptor, at their origin long ago we might have imagined how they would develop; but unlike tables and statues they never turned out as we expected. And, yes, as they changed we tried to control them, but we didn’t always succeed. Further, while they weren’t planned, they still developed seeming order.
Where, though, did that order come from? There Aristotle was truly stuck. Perhaps that’s why he had to imagine that a politeia, a soul, imposed it. That was the only way he could make everything fit because in his cosmos, everything that existed must have always existed. So, for him, whatever perceived purpose anything had now, it must have always had.
Thus, were we to ask him why polar bears have fur, he would say that their fur protects them from cold—that is, their fur is for the purpose of warmth. But it never occurred to him that if the arctic were to warm up, that same fur would still retain heat, yet its apparent purpose would then be to give them heatstroke. In his cosmos, the planet could change, but not the life-forms on it.
Lucretius, born in Rome two centuries after Aristotle died in Greece, avoided that problem. He would have said that order came not from divine intent or inbuilt purpose but from sheer chance. He wrote that “Nothing originates in the body [in order] that we may use it, but once originated, we find a use for it.” So he, and others like him, turned Aristotle’s idea of built-in purpose on its head.
For Lucretius, polar bears wouldn’t have fur because unseen somethings inside them caused them to have it (to keep themselves warm). Rather, those with no fur likely died young; so the ones that we see today are furry. But he never said how they came to be in the first place, just as Aristotle never said how their built-in purposes could come to change.
Darwin, nearly two millennia later, found an explanation for the order we see in life-forms. He said that living things, at least the things that we today agree to call ‘alive,’ are those things that can copy themselves (or things, like mules, that arise from things that can copy themselves). They might make many such copies, and while those copies are like them, they aren’t all the same. Further, variation among copies affects survival, and not all copies can persist long enough to make more copies because resources are limited. So some varieties persist while others don’t. Mix copying with variation and add limits to give evolution. Shake and bake for a few billion years and out comes us. (Or whatever else may have come out of the same oven if the Earth did the experiment again....)
Darwin’s idea explained a lot of stuff, and it was testable. So even before we knew about genes, it began to compete with our older ideas of divine intent, inbuilt purpose, or sheer chance—but only for what we agreed to call living things—that is, integral masses of goo that could copy themselves. What, though, about the order we see in everything else? Also, to get Darwin’s process started we needed life. But where did life itself come from?
Complex networks, like life itself, are so complex that they appear designed, but they appear too complex to design. They aren’t alive the way kittens are, so Darwin’s scheme doesn’t seem to apply to them. However, they act at least a little bit as if they are in some way ‘alive.’ Their structure and behavior seem too intricate and purposive to have arisen by sheer chance. So Lucretius’ scheme doesn’t seem to apply, either. But Aristotle’s scheme also doesn’t seem to apply. They usually have no rulers (or politeia); or if they do, which means we’re involved, while our plans can matter, we never fully control them.
So if a complex network isn’t ‘alive,’ but appears to have purpose—yet if no entity may have planned to put that purposiveness there before the network came to exist, how then did it get there? Do we have to fall back on divine intent?
Swarm physics suggests another possible explanation: Maybe such networks put purpose there themselves.
Here’s how: New things try to enter complex networks all the time. It might be a new gene in a cell, a new termite in a termite colony, a new plant in a food web, a new building in a city, a new firm in an economy, a new word in a language, a new law in a legal code, a new tool in our swarm. Whatever it is, it’s constantly faced with threats to its persistence as the network’s nodes react to it. It doesn’t matter if it’s made of chewing gum or titanium, or if it’s as small as a molecule or as large as a city. It will die out unless some subset of the existing network feeds it, protects it, maintains it, reinforces it, or makes copies of it. If that happens, though, it stops being a new thing and becomes just another node in the network.
A twist on an old physics fable suggests how that might happen. Suppose that after the first barometer was invented someone were asked to use it to measure a building’s height. The expected answer is to measure air pressure on the ground, measure air pressure on the top of the building, then use the difference to work out the height. But another way is to stand the barometer upright on the ground, measure its shadow, measure the building’s shadow, then use similar triangles to find the building’s height. Or: offer the barometer to the building’s janitor in exchange for the building’s height. Or: sell the barometer and buy a tape measure. A barometer may have many uses—or no use; that depends on the network it lands in, and when it lands there. Its inventor may be thinking of air pressure, but we may just think it’s pretty and nail it to the wall as a piece of art.
Every network invader costs something to build—and perhaps even more to develop in the first place. For it to persist in the network, it has to find a use. How its copies come to get made, and how they come to get used, is up to the structure of the network it lands in, and when it lands there. (For such networks, location is important, but so is timing; they have geography and history.)
That’s true even if the invader can copy itself. A dandelion seed can’t persist if it invades where there’s no humus—or after saplings have sprouted, since it would then have no sunlight.
Further, for such a network to be able to make copies of any successful invader, before invasion it had to have recursive closure. That can happen had it grown ecogenetically. Its reactions would then have already had recursively self-reinforcing structure—or else it wouldn’t have persisted long enough for the new thing to enter it in the first place.
So a successful invader of a complex network tends to be one that happens to fit into the invaded network at that particular place at that particular time, like a key in a lock. Had it invaded the same network but somewhere else—or somewhen else—it may well have died out. This isn’t ‘survival of the fit’ but ‘survival of those that fit.’
Thus, at least for complex networks, an invader adapts to the network it invades—or not. But if the invader does adapt, then so does the network. That’s because for an invader to become a new node in a network, its entrance must trigger new reactions that will support it. But those reactions will trigger changes in preexisting reactions, which trigger changes in yet other reactions. That deforms the network. Thus an invader’s successful entrance drives down support for some current nodes and drives up support for some future possible nodes. After an absorption lag, the network as a whole regains recursive closure, becoming not the old network plus an invader, but a new network. It’s only a figure of speech to say that it’s the ‘same’ network, whether cell or nation.
So, the network chooses; but if it does, that then changes it. The invader might even go on to become a hub of the new network—the center of a swirling cluster of focused nodes, linked to other hubs in other clusters of nodes doing related things.
That’s what seems to have happened with the steam engine. On creation, it had only very narrow use and could easily have died out. Over time, though, it found wider and wider use until many reactions already in the network linked to it. (However, as the printing press suggests, that happenstance depends on when and where it hits.) Ditto for the railroad, and again for mass production. Perhaps something similar happened in protocells with ATP billions of years ago, then later with kinesins, and again with mitochondria—and all the other pieces that make up the various kinds of cells lying around the planet today.
So while every successful invader deforms the network, almost all deform it only a little, while a few—ATP, kinesin, the steam engine, the railroad—go on to do so deeply.
Over time, after many invaders, few of which might become new nodes, and even fewer of which might become new hubs, the network becomes very dense and very diverse—clusters of interlocking clusters. Unless, of course, it’s hit by too disruptive an invader, or too many invaders at once, in which case it may degrade, or dissolve entirely.
The central principle, then, behind a sufficiently complex network seems to be that links between its nodes must be both very dense and also widely diverse. Every node in it is there for a reason—and, usually, many reasons. Those reasons depend on the history and geography of prior invasions, including not just what invaded but their specific sequence and location of invasions. In such a network, a few nodes are hubs—they link to many others nodes—while most nodes may link to only a few—yet all nodes are necessary (or the network falls apart). Also, linkage strengths between nodes may vary widely—some nodes may link strongly while others link only weakly. And what nodes are there, how many there are, what links to what, and how strongly they link, all may vary over time.
At first glance, that such a complex thing could arise, far less persist for any length of time, as a possible outcome of a mere pinball game of random events seems startlingly unlikely. But maybe that just depends on how long the game goes on.
However, there also needs to be an abracadabra step: If such a network were to persist long enough, then an integrated whole that maintains itself and some sort of boundary might emerge. It happened with cells billions of years ago; it happened with termites millions of years ago. How that happened in either case, we still don’t know; we haven’t done it in the lab yet. But however that happens, the network would then have an inside and an outside, and might even start compartmentalizing further.
If such a buildup could happen by itself, no single entity need necessarily be making the resulting network’s ‘decisions,’ nor need any entity have planned it beforehand, nor need it even be plannable in principle. The network as a whole might do it. But if it does, that needn’t mean that such decision-making is random. If the network doesn’t vet potential invaders, then, like its failed invaders, it, too, will die out.
In short, any thing (and any reaction it triggers) that tries to invade a sufficiently complex network, but which, for whatever reason, doesn’t catalytically fit into the network at just that place and just that time, will die out. And any network that doesn’t screen invaders (and the reactions they trigger) for that particular sort of fitness likely won’t grow to become sufficiently complex, and thus will also die out. So, factories and the networks of factories that they fit into would themselves be much like parts and the factories that make them—they depend on each other for their persistence.
Thus, when we look around, rarely would we see such maladroit parts, or such inept networks. In time, they would all die out. The parts would compose the current network, but also the current network would select its future parts. Perhaps that’s how the whole can become more than the sum of its parts. Perhaps that’s even what makes a complex network complex.
So when we try to change a complex network that seems to be just a lot of unrelated, higgledyâpiggledy bits and pieces, it resists—either returning to its previous state, or transforming into some new but equally persistent state. It’s resilient (that is, it tends to be hard to disturb), resistant (that is, once disturbed, it tends to return to its previous state), and recursive (that is, all its parts tend to link to many other parts).
Since it’s robust, and intricate, and it actively resists dissolution, it’s hard not to think of it as, in some sense, purposive—intent on going its own way and doing its own thing. Somehow it persists; but not necessarily because anyone designed it to be that way. It may be the way it is just because a long stream of invasions continually deformed it by reconfiguring its nodes to support each other—usually in very small steps over very long periods.
Of course, that chain of reasoning is very loose, but if it could one day be firmed up then perhaps we might begin to answer Aristotle’s original question about why things are the way they are this way: at least some of the order that we see around us might be neither inborn nor inbuilt. It might be ingrown. If so, both Aristotle and Lucretius were right, in a way: ‘purpose’ can become baked in to at least some persisting networks—but not at random, nor all at once, nor before the start, nor necessarily by anyone’s intent. It might get baked in because, over time, all networks without it cease to persist. In a sense, some networks can grow to be so complex that they can cause much of their own behavior.
When it comes to a network that we’re a part of, we may try to drive it this way or that, but, like trying to tame an ornery steer, that needn’t mean that it will do what we wish (assuming we all ever agree on anything). Nor need it mean that if it doesn’t do what we want then it must thus be behaving randomly. It may behave non-randomly, yet not the way we wish. In that sense at least, it may seem ‘alive.’ If so, we aren’t careening into our future at random, but we also aren’t in control. It is.
Were we to accept that, then, in some sense, the central question we might each ask ourselves is unanswerable, but maybe is still worth asking; it would be like a protein in a cell, or a termite in a colony, asking itself something along the lines of: Is there Something Else here? If so, does It know more than we know, or less? And even more importantly: does It know what It’s doing? ’Cause we sure don’t.
Of course, that long chain of thought isn’t science. It’s not mathematically specific, nor is it testable. It’s not yet a theory. It isn’t even a hypothesis, quite. So maybe it’s yet another handwavy just-so story that can be twisted to explain anything, in which case it explains nothing. However, if it can be said to have a crude hypothesis that might be relevant to us, that hypothesis is this: The tools we invent drive us to act together, and over longer and longer distances in spacetime. That happens even when we don’t wish it, and even if we don’t see it. It’s not something we have to plan or foresee or intend; it’s not necessarily to our individual benefit; and it may even be to our species detriment in the long term. But over time, we seem to act more and more as one entity. Ultimately, that seems to be why we are able to do what we do.
When we look around, we see many highly ordered things, and many of them are networks. Why? Both the Greek and the Roman say plausible things about order, yet neither view seems to explain much about the origin of order in networks. Their various devotees have been quarreling about who’s right for over two millennia now. ‘Purpose!’, some shout. ‘Chance!’, others yell. But their views are just two ways to state the same fact, namely: Order exists. Whether it explains more to view that fact through Aristotle’s or Lucretius’ eyes is a question for philosophers. For scientists, though, the question of why there is order is far too hard. The question of how that order might have come to be is already hard enough.
Aristotle’s way of thought is still worth examining today because it’s still common among us. Perhaps that’s because through, or behind, or under, all the chaos around us, we see rules, and whenever we see rules, we assume a rule-maker. Apparent design, whether in a rosebud, a flock of geese, a school of fish, or an economy puzzle us if we see no obvious designer. Further, demonstrating that built-in purpose needn’t always be necessary to produce order isn’t the same as demonstrating that it doesn’t exist. For Aristotle, the cosmos must not only have meaning, it must have meaning for us. So answering each of his successive ‘whys’ about railroad networks or cities or economies or whatever with increasingly intricate ‘hows’ might interest him, it might intrigue him, it might even astonish him, but it might not satisfy him. The how of something can tell us a lot, but it doesn’t tell us the why.
Thus, it seems possible that many of us don’t really wish to know how a thing works, or what it’s made of, or when it may have come to be. That’s for geeks. We want to know why it exists. We want to know what it is for. But science can’t answer such a question anymore than it can say what a sunset or a kitten is for, because such a question isn’t really about sunsets or kittens at all but a question about us, and why (or rather, if) we matter in the cosmos. Thus, for many of us outside science, asking ‘why’ seems quite reasonable. We’re then often not really asking about cause, but intent—and therefore Aristotle’s same old desires: ends, goals, agency, planning, purpose. That’s how we’re used to thinking about the cosmos, perhaps because that’s how we’re used to thinking about each other. Our drive to seek the ‘why’ may thus not really be a request for explanation, but a disguised plea for meaning—that is, purpose we can understand. Maybe we can’t accept that, to us at least, the cosmos may have no meaning at all. There may be no why.
No doubt you’ve probably spotted the problem with this maniac—possibly megalomaniac—mapping between biomolecular and entomological networks and human networks. It’s trying to use one thing we don’t understand to explain another thing we don’t understand. It’s a mere analogy, not science. We aren’t molecules; termites aren’t people. So as a poet said 2,500 years ago, it’s “but a woven web of guesses.” However, even metaphors can sting, just as some bees can. It does seem as if there is such a thing as a ‘swarm’ for biologists to study, just as much as there is an ecology for ecologists to study, and maybe even as much as there is such a thing as an economy for economists to study. Considering it to be ‘alive,’ in some sense, might be reasonable, too. Whether we form one, though, is not so clear—that might be just another mirage. But if we do form one, it does seem as if at least some part of how we work and where we’re headed is due more to it than either sheer randomness or anything we intend. If that’s so, figuring out more about how it works might be a useful thing to try to do.
Finally, saying that our swarm is alive is a little like saying that a tank is a heavily armed recreational vehicle. Fine. But what we really would want to know is: what would that mean for the tourism industry? There are always going to be folks running around waving placards with Vague Theories Explaining Everything* (*Except For All The Stuff That Somehow Doesn’t Fit). So you’re probably pretty suspicious. You’re right to worry. We have a word for folks who claim to explain the whole world with just one idea—they’re called cranks. Our swarm can’t explain everything about us. The world is too complicated. So forget about the philosophy flapdoodle for now. What might thinking of ourselves as parts in a swarm mean for our future? If nothing else, it seems to suggest that we’re parts of something that is becoming, or maybe even has already become, a self-maintaining network—a highly intricate, highly reactive, fast-acting planetary entity. That suggests that the faster our future changes come, the less likely are we to be able to predict them, or be even remotely in control of them. If so, changes in our swarm might come less and less from our intentions and more and more from the demands of its own structure. In short, perhaps, our swarm is now so dense and diverse that it’s nearing another big phase change—but into what we don’t know. Perhaps we may be discovering our swarm too late for that knowledge to be truly useful.